2023 Eilers Scholars
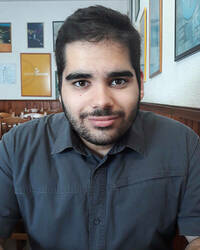
João Dinis Oliveira Abranches
Chemical and Biomolecular Engineering
Faculty Advisors: Yamil J. Colón and Edward J. Maginn
Novel Sustainable Materials Design with Thermodynamics-Informed Machine Learning
Rare earth elements are a set of seventeen chemical elements that play a central role in several vital technologies (e.g., fuel cells, electronics, and catalysis). However, there is a lack of efficient and sustainable processes to extract and refine rare earth elements, which has driven several regulatory agencies, including the US Department of Energy, to classify them as critical materials. Attempts at designing new solvents for their extraction have traditionally followed trial-and-error methodologies, often complemented by basic heuristic rules or chemical intuition (e.g., “like dissolves like”). To date, this simplistic approach has led to the discovery and characterization of only a small fraction of all synthesizable compounds. This limitation is aggravated when studying mixtures, as the number of all possible molecule combinations is virtually infinite. The massive amount of resources and extreme cost necessary to explore this chemical landscape is simply too demanding to be feasible. Data-driven methods such as machine learning are promising alternatives to these trial-and-error methodologies. However, they are severely hampered by the need to represent materials in a machine-intelligible way (i.e., converting molecular structures to sets of numerical values that can be understood by mathematical machine learning models). In fact, most molecular representations proposed to date lead to overly complex models that require a tremendous volume of experimental data to be properly trained.
To bridge the gap between small, scarce datasets and data-driven approaches, this project aims at designing novel sustainable materials using thermodynamics-informed machine learning models, with a particular focus on solvent mixtures for the extraction and refinement of rare earth elements. The use of thermodynamics information will be achieved in two separate ways. The first is to use correlative machine learning methods, namely active learning, to describe activity coefficients (a single property), which can then be combined with thermodynamic models to predict a plethora of different solvent-related properties (e.g., phase equilibria and solubility). The second thermodynamics-informed approach is to develop molecular descriptors that encode thermodynamic information, namely molecular polarity, and that possess a small, fixed size. This will greatly limit the number of trainable parameters of machine learning models, particularly deep neural networks (DNNs), and, thus, reduce the size of the datasets necessary to train them. Finally, inverse design workflows based on this type of thermodynamics information will also be constructed, which will enable the computational design of tailor-made materials bounded by a set of physicochemical properties selected based on a given application of interest.
Research ObjectivesThe results of this project will constitute a major breakthrough in the way novel materials can be designed, as the machine learning models developed are expected to be robust, accurate, and generic enough to be applied across several families of compounds and materials. The preliminary results obtained thus far support this vision, as they showcase, for example, the remarkable ability of sigma-profile-based DNNs (a molecular descriptor combined in this project, for the first time, with deep learning) to accurately correlate and predict a wide range of solvent-related physicochemical properties. Moreover, the correlative machine learning approaches developed can describe the phase equilibria of a plethora of relevant systems, such as deep eutectic solvents, single-ion activity coefficients, and ternary systems, using, in many cases, as little as one experimental data point.
Objective 1: Thermodynamics-Informed Active Learning. The first objective of this project is to describe the activity coefficients of liquid mixtures using Gaussian Processes (GPs) and active learning as correlative tools. Activity coefficients are crucial to model phase equilibria and, thus, are essential to design pathways for the recovery of rare earth elements, as well as to minimize the energy requirements of unit operations (e.g., distillation evaporation, crystallization, absorption, solubilization, and liquid-liquid extraction). Emphasis will be given to multicomponent systems where ionic liquids behave both as extractants and dilutants, and that display a certain degree of immiscibility with water. These systems are critical in the extraction and processing of several metal ores, namely those that contain rare earth elements, and are solely governed by activity coefficients.
Objective 2: Thermodynamics-Informed Deep Learning. Sigma profiles (unnormalized histograms of screened charges, obtained using quantum chemistry calculations), being a thermodynamics-informed molecular descriptor, provide several advantages over classical molecular representations in deep learning. This has been verified by us in a previous publication [1], where sigma-profile-based deep neural networks were able to accurately correlate and predict the molar mass, boiling temperature, vapor pressure, density, refractive index, and aqueous solubility of a myriad of organic compounds. This framework will be now expanded to encompass the design of deep eutectic solvents and ionic liquids, which are sustainable materials with tremendous energy-related applications. Moreover, the absence of open-source tools available in the literature to generate sigma profiles will be mitigated by building a Python tool that interfaces with RDKit and the open-source quantum chemistry package NWChem.
Objective 3: Thermodynamics-Informed Inverse Design. The final objective of this project is to enable the inverse design of novel sustainable materials by combining the active learning and deep learning frameworks developed in the two previous objectives. The hallmark of inverse design is the ability to directly obtain molecular structures that are predicted to possess a given set of properties (e.g., properties that are attractive for sustainable solvents). This will be achieved by developing a sigma-profile-based encoder/decoder, which has two main functions. First, the encoder will bootstrap the development of sigma profile databases. Instead of relying on expensive quantum chemistry calculations, the encoder will be able to directly generate sigma profiles from graph molecular structures, decreasing the computational cost of building vast sigma profile datasets. Second, inverse design will be enabled by coupling the decoder with the DNNs developed in Objective 2. That is, a sigma profile, which has been identified as maximizing a particular target property, can be fed into the decoder to obtain its corresponding molecular structure. Having developed this encoder/decoder, the navigation of the sigma profile space will entail the use of active learning (more precisely, Bayesian optimization) to obtain sigma profiles that match a set of criteria to be appliable as sustainable materials.
[1] Abranches, D. O.; Zhang, Y.; Maginn, E. J.; Colón, Y. J. Sigma Profiles in Deep Learning: Towards a Universal Molecular Descriptor. Chem. Commun. 2022, 58 (37), 5630–5633. https://doi.org/10.1039/D2CC01549H.
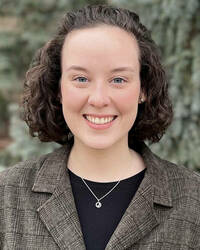
Carmen Chamberlain
Chemistry and Biochemistry
Faculty Advisor: Peter C. Burns
High Pressure Studies of Uranium (VI) Minerals to Promote a Safer and More Sustainable Future for Nuclear Energy
Until battery science has progressed enough to make solar and wind energy consistently available to meet baseload needs, nuclear energy is the most reliable and low-carbon source of sustainable energy available. However, the treatment and disposal of nuclear waste is complicated by its propensity to change composition over time and the potential hazards it poses to the environment. In the United States political indecision over where to locate a centralized repository for used nuclear fuel has led to dry storage on-site at each plant. Because this storage is not meant to be permanent, the potential for failure of outer containment and contact with the environment increases with time. When used fuel pellets were exposed to water from Yucca Mountain, the proposed repository site for energy-related nuclear waste, the uranyl sheet minerals boltwoodite and uranophane formed.[1] These sparingly soluble alteration phases limit the transport of uranium and certain cations in the environment, and they are likely to incorporate various fission products and transuranium elements, thereby slowing radionuclide mobility. Consequently, a better fundamental understanding of the structural stability of these phases would advance uranium chemistry overall while providing specific information related to the potential mobility of uranium, fission products, and higher actinides incorporated into these mineral phases. The goal of this project is to characterize pressure-induced structural changes of uranyl minerals relevant to nuclear waste to further the fundamental understanding of uranium chemistry for safe storage and treatment of used nuclear fuel.
High (gigapascal range) pressure studies are often used to elicit phase transitions in crystalline material such as uranium dioxide.[2–4] While these transitions typically occur at pressures greater than 20 gigapascals,[2–4] more subtle structural changes such as an induced agostic interaction in a uranium (IV) organic structure occur at pressures below 4 gigapascals.[5] Low gigapascal-range pressures are geologically relevant, and eventual collapse of components of a mined geologic repository, such as Yucca Mountain, will result in increased pressures over ambient. Gigapascal range pressures are achieved experimentally using diamond anvil cells (DACs) which greatly limit the number of compatible analytical methods due to the mechanical support necessary to generate such high pressures. Two commonly used methods with DACs are single crystal X-ray diffraction to determine atomic positions and Raman spectroscopic measurements to characterize short range order and changes in symmetry within the materials.
Foundational ObjectiveObjective 1: Develop and optimize methods for high pressure single crystal X-ray diffraction (SC-XRD) and Raman spectroscopic data collection for uranyl minerals. The primary challenges thus far have been 1) the limited range of SC-XRD data that can be collected due to the geometry of the DAC, and 2) incompatibility between certain samples and the hydrostatic pressure mediums (used to ensure force is applied equally in all dimensions) we have readily available. A natural sample of boltwoodite ((K0.56Na0.42)[(UO2)(SiO3OH)](H2O)1.5) has been used to optimize SC-XRD methods because of its compatibility with the hydrostatic pressure medium 4:1 methanol to ethanol. Recently we completed the first phase of experiments and verified that uncertainties in bond lengths are dictated by disorder of the structure rather than the limited geometry of the DAC data collection. Further experiments to this end are currently underway. The second challenge remains a limiting factor, but we continue to test minerals of interest with other hydrostatic pressure mediums to overcome this issue.
Proposed ObjectivesObjective 2: Apply high pressure methods to other uranyl silicate minerals known to form on used nuclear fuel pellets under repository conditions. Direct comparisons can be made between boltwoodite and α-uranophane (Ca[(UO2)2(SiO3OH)2](H2O)5) that share the same uranyl silicate sheet structure aside from the interlayer cations. This comparison will begin to establish the significance of interlayer cation identity on the stability of the structure. Soddyite ([(UO2)2(SiO4)](H2O)2) is a uranyl silicate that forms a framework without charge balancing cations. I hypothesize the interconnectedness of the soddyite structure will cause it to be significantly altered to the point of losing crystallinity or undergoing a phase transition at lower pressures than boltwoodite and α-uranophane due to the strain having no other way to be relieved.
Objective 3: Apply high pressure methods to minerals with similar sheet structures and the same cations as boltwoodite/uranophane. This would allow for the comparison of the stability of mineral species based on the oxyanion involved in the structure and allow for trends in the interlayer cation composition to be established. Understanding the influence of the interlayer cation on the structure’s stability will help to predict how well fission products may be immobilized in the structure. The results of this comparison would be particularly useful if a new geologic location with different geological composition is considered as a potential repository site.
Objective 4: Determine the basis of incompatibility of certain minerals with the hydrostatic pressure medium 4:1 methanol to ethanol. Preliminary high pressure work with α-uranophane has been limited by seeming incompatibility with the hydrostatic pressure medium that is unexpected since boltwoodite and α-uranophane share the same sheet structure. The interlayer includes water in both minerals, and I hypothesize the hydrostatic pressure medium more easily displaces the water associated with Ca2+ in α-uranophane than the water associated with Na+ and K+ in boltwoodite. Understanding this incompatibility would further the goal of stability comparison of these minerals and may highlight the importance of interlayer cation as well as connectivity that could be verified in high pressure experimentation.
[1] Finn, P. A.; Buck, E. C.; Hoh, J. C.; Bates, J. K. Spent Fuel’s Behavior Under Dynamic Drip Tests; Argonne, IL, 1995.
[2] Idiri, M.; le Bihan, T.; Heathman, S.; Rebizant, J. Behavior of Actinide Dioxides under Pressure: UO2 and ThO2. Phys Rev B Condens Matter Mater Phys 2004, 70, 014113-1-014113–014118.
https://doi.org/10.1103/PhysRevB.70.014113.
[3] Gréaux, S.; Gautron, L.; Andrault, D.; Bolfan-Casanova, N.; Guignot, N.; Haines, J. Structural Characterization of Natural UO 2 at Pressures up to 82 GPa and Temperatures up to 2200 K. American Mineralogist 2008, 93, 1090–1098. https://doi.org/10.2138/am.2008.2735.
[4] Livneh, T.; Sterer, E. Effect of Pressure on the Resonant Multiphonon Raman Scattering in UO2. Phys Rev B Condens Matter Mater Phys 2006, 73 (8), 085118-1-085118–085119.
https://doi.org/10.1103/PhysRevB.73.085118.
[5] Arnold, P. L.; Prescimone, A.; Farnaby, J. H.; Mansell, S. M.; Parsons, S.; Kaltsoyannis, N. Characterizing Pressure-Induced Uranium C-H Agostic Bonds. Angew. Chem. 2015, 54 (23), 6735–6739. DOI:
https://doi.org/10.1002/anie.201411250.
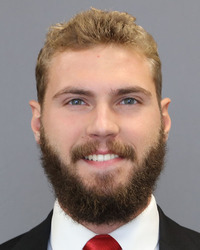
Russell Clarke
Chemical and Biomolecular Engineering
Faculty Advisor: Jason C. Hicks
Low-Temperature Dehydrogenation and Coupling of Light Alkanes using Nonthermal Plasma-Assisted Catalysis
The recent surge in US shale gas production has been the largest development in energy of the 21st century [1]. The resulting excess of light alkanes and the economic challenge of transporting gaseous hydrocarbons from remote well sites have caused over 500,000 Mcf per year of unused shale gas to be vented or flared nationally, corresponding to 10^7 tons of CO2 unnecessarily released to the atmosphere [1]. This underutilization of a valuable resource has created an engineering opportunity to develop a modular process that can use renewable energy to reform the shale gas to condensable hydrocarbons (C4+), which are more easily stored and transported. This work proposes using an electrically driven nonthermal plasma coupled with a heterogeneous catalyst to activate the C-H bonds in the shale gas and selectively form more desirable products, such as olefins or long-chain hydrocarbons. Nonthermal plasmas are generated by applying a strong electric field across a gas, which ionizes the gas and accelerates free electrons to very high energies on the order of 10,000 K while maintaining a bulk-gas temperature near 300 K [2]. The high-energy electrons can then interact with other gas-phase species in numerous ways, creating a complex mixture of ions, free electrons, excited species, and free radicals that react with greater activity than what is accessible thermally at near-ambient conditions. Because the transient species formed in the plasma are not in equilibrium, this technique is capable of achieving reactant conversions beyond equilibrium limitations, which makes endothermic reactions like ethane and propane dehydrogenation ideal targets for this process [3].
Furthermore, addition of a catalyst bed to the plasma discharge region has been shown to enhance the activity of the plasma under specific conditions [4]. At ambient temperature and pressure where the thermal activity is negligible, preliminary results with ethane (the second largest component of shale gas behind methane) show a 53% increase in ethylene production rates when a commercial PtSn bimetallic dehydrogenation catalyst was added to the packed bed in the plasma reactor. While similar results have been explained by the enhanced electric field in a packed bed or resistive heating of the catalyst nanoparticles by the plasma, recently published work on plasma-assisted catalytic nitrogen fixation indicates that there is a lowered nitrogen activation barrier due to direct interactions between plasma-excited species and the catalyst active site [5]. Because it is unknown what is the dominant process for plasma-assisted alkane dehydrogenation, a major focus of this work will be to determine the origin of the plasma-catalyst synergy by systematically eliminating competing hypotheses (e.g., minimize catalyst heating by operating with a cooling jacket at sub-ambient temperatures). Finally, investigating the stability of bimetallic catalysts in plasma conditions will be an additional focus of this work. Because bimetallic catalysts have low thermal stability and plasma operates at low bulk-gas temperature, we hypothesize that thermally driven catalyst deactivation processes such as sintering, coking, and leaching will be minimized during plasma operation, increasing catalyst stability. Support for this hypothesis may also lead to future work in plasma regeneration, where an oxygen plasma may be able to remove coke without requiring high temperatures that cause sintering and leaching.
Research ObjectivesObjective 1: Quantification of Plasma Influence. Starting with an ethane feed and moving to more complex propane and mixture feeds (hydrogen, methane, ethane, and propane at simulated raw shale gas compositions), this objective will measure alkane conversion and olefin selectivity at different plasma power, flowrate, feed composition, catalyst loading, and bulk-gas temperature. The experimental results obtained in this study will be supplemented by a microkinetic model built using ZDPlasKin software to inform dominant reaction pathways in the plasma phase and predict conditions that will enhance either dehydrogenation or C-C coupling reactions.
Objective 2: Informing Catalyst Selection Criteria. Once the contribution of the plasma is understood from Objective 1, the contribution of the catalyst can be studied in context. Therefore, Objective 2 is to seek insight into the catalyst properties that yield favorable activity, selectivity, and stability under plasma exposure, and determine the origin of the observed plasma-catalyst synergy. Objective 2 is rooted in the postulate that the most active thermal catalyst is unlikely the most active plasma catalyst due to differences in reactant binding energy [5]. Therefore, the industrially used PtSn on alumina dehydrogenation catalyst will be investigated first to limit unknowns, then the primary metal will be exchanged and tested to determine trends or optima in activity with binding energy. This objective will also investigate catalyst mixtures (e.g., PtSn/alumina with Ni-BEA) designed to perform dehydrogenation to olefins and subsequent oligomerization to C4+ hydrocarbons.
Objective 3: Design and utilize an in situ diagnostic approach to observe plasma-catalyst interactions. The first part of this objective is to design an infrared transmission spectroscopy tool that is capable of characterizing the surface of catalysts and support materials under non-thermal plasma stimulation (completed, ref. [6]). This technique will then be used to support Objective 2 by probing the surface of different catalysts under ethane and propane plasma stimulation to quantitatively detect adsorbed species and compare the surface coverages between catalysts and during thermal and plasma operation.
[1] Wang, Q.; Chen, X.; Jha, A. N.; Rogers, H. Renew Sust Energ Rev 2014
[2] Bogaerts, A.; Tu, X.; Whitehead, J. C.; Centi, G.; Lefferts, L.; Guaitella, O.; Azzolina-Jury, F.; Kim, H. H.; Murphy, A. B.; Schneider, W. F.; Nozaki, T.; Hicks, J. C.; Rousseau, A.; Thevenet, F.; Khacef, A.; Carreon, M. J Phys D Appl Phys 2020
[3] Mehta, P.; Barboun, P. M.; Engelmann, Y.; Go, D. B.; Bogaerts, A.; Schneider, W. F.; Hicks, J. C. ACS Catal 2020
[4] Kim, J.; Abbott, M. S.; Go, D. B.; Hicks, J. C. ACS Energ Lett 2016
[5] Mehta, P.; Barboun, P.; Herrera, F. A.; Kim, J.; Rumbach, P.; Go, D. B.; Hicks, J. C.; Schneider, W. F. Nat Catal 2018
[6] Clarke, R. J.; Hicks, J. C. ACS Eng Au 2022
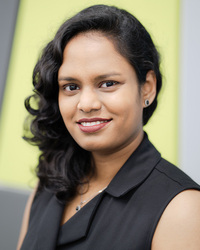
Wedage Lakna Nuwanthi Dayaratne
Chemistry and Biochemistry
Faculty Advisor: Adam Jaffe
Hybrid Molybdenum Bronzes for Energy-Related Applications
Crystalline metal oxides are an inorganic class of materials that show a wide range of chemical and thermal stability. Once reduced, some metal oxides—often referred to as bronzes due to their metallic luster—take the form AxMO3 (A = cation, M = metal) and display favorable electronic properties for applications ranging from energy storage to catalysis. These inorganic solid-state materials, however, can require high-temperature syntheses and are difficult to tune post-synthetically. To address these challenges, we are taking initial steps toward the development of a class of materials we call “hybrid bronzes” that combine the favorable structural, redox, and electronic properties of extended inorganic bronzes and the tunable functional groups and synthetic control of the organic molecules.
Our specific aim is to template two-dimensional layered hydrogen molybdenum bronzes with molecular species to modulate their electronic properties. The variable oxidation states of molybdenum and the tunable band gap and facile charge transport associated with reduced MoO3 has made molybdenum bronzes strong candidates for numerous applications such as in electrochromic devices and catalysts. In preliminary work, we have generated a library of such reduced all-inorganic molybdenum oxides as well as their hybrid congeners by following mild, solution-state reaction conditions. We have also begun examining their structure-property relationships utilizing powder X-ray diffraction, vibrational and electronic spectroscopies, and electrochemical methods. These designer materials will be subjected to conductivity measurements and electrochemical analysis to observe how molecular templating effects change electronic properties. This includes investigation of electronic band gap tuning and study of these materials’ mixed electronic and ionic conductivity. We are particularly interested in utilizing the unique tool of high-pressure experimentation to understand stimulus-induced changes and how structure dictates the electronic behavior of these materials. These materials seek to address the world energy crisis by providing a tunable material platform. We aim to gain fundamental insight into structure-property relationships within these systems and investigate the potential application of these materials toward battery electrodes, electrochromic devices, pressure-induced switching devices, and catalysts.
Research ObjectivesThe overall goal of this project is to design a new material platform of organic-inorganic hybrids that have the potential to address energy-related applications. Specific major objectives are:
Objective 1: To develop multiple robust synthetic approaches toward hybrid molybdenum bronzes. We will follow two main synthetic methods to introduce organic species within the layered architecture: (1) intercalation and (2) templation. Intercalation is the process of reversibly inserting the organic ligands without disrupting the underlying geometry and topology of the material. Templation is a phenomenon where precursor species self-assemble into the final hybrid architecture under the structure-directing behavior of the organic ligands themselves. These two synthetic strategies result in different connectivity within the materials and thereby would change their inherent optical, electronic, and structural properties, including modulation of band gap and electron mobility.
Objective 2: To probe how the electrochemical behavior of our hybrid systems varies with molecular properties such as steric bulk, hydrogen-bonding, and charge density. Our preliminary results have indicated a significant change in the redox potentials for hybrid structures depending on the organic group that is incorporated. We will first assess these structural-electrochemical correlations by systematically varying the above-mentioned molecular features via ligand choice and observing how this affects the electrochemical response. Next, we will introduce redox active molecular species into the hybrid structure to enhance access to varied oxidation states, allowing for greater capacity in energy storage applications or improved catalytic functionality. In promising initial results, we have accessed multiple redox states in organic ligands by using redox active species such as 4,4’-azopyridine and we hope to expand this molecular phase space with other redox and photo active ligands. The goal is to observe charge delivery between the inorganic layers and the organic ligands, thereby demonstrating the synergy of good charge transport pathways with chemically functional molecules. These electrochemical measurements will allow us to prioritize suitable candidate materials that exhibit good conductivity and electrochemical reversibility for further study.
Objective 3: To understand and control charge transport within these hybrid bronzes. The level of reduction of molybdenum oxide has a marked impact on the conductivity of the material, but little is known about how the introduction of organic species will affect charge carrier mobility or if it will allow for greater property control. In promising preliminary results carried out with our in-house potentiostat, we have demonstrated that the introduction of organic species only decreases conductivity by one order of magnitude despite the dramatic restructuring that it causes. In other words, we can retain the conductivity of the material while enhancing the properties of the bronzes with the introduction of organic ligands that can be selected to tune charge transport. By varying reduction levels of the molybdenum bronze, introducing additional redox-activity via the organic species, and positioning organic and inorganic species in close proximity to allow for charge transfer, we have many handles for tuning charge transport.
Objective 4: To utilize pressure as a fundamental tool to probe structure-property relationships. By incrementing the pressure we are able to access parts of the thermodynamic phase space that are not accessible under ambient conditions. These states often have different connectivity, band gaps, and conductivity and can therefore direct future synthetic modifications of these materials toward given applications. The orbital overlap with be systematically studied under the variation of pressiure. Since pressure can be leveraged as a stimulus to induce electronic property changes within these materials, this even implies the possibility of a next-generation switching paradigm for information processing technologies. Key challenges include precise tracking of phase changes under pressure and following oxidation state changes, which we will accomplish through a variety of in situ diffractive, spectroscopic, and electrical transport measurements.
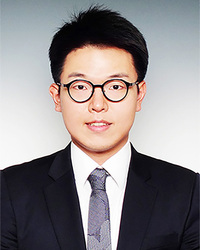
Garam Lee
Chemical and Biomolecular Engineering
Faculty Advisors: Casey O'Brien and David Go
Understanding the formation and reactivity of metastable azide (N3) on metal surfaces from the interaction with non-thermal plasma-activated nitrogen (N2)
Synopsis: The goal of this research is to understand and explore a new approach to the formation and utilization of metastable azide (N3) on metal surfaces for nitrogen fixation. The importance of this research is to unambiguously identify the surface-adsorbed nitrogen species and its formation mechanism when metal surfaces interact with nitrogen (N2) in the presence of non-thermal plasma (NTP). If successful, this research will have guided a novel way for nitrogen fixation.
Motivation: Non-thermal plasmas (NTPs) are partially ionized gasses in which high energy electrons collide with gas molecules to produce a highly reactive chemical environment, including free electrons, ions, radicals, and vibrationally- and electronically-excited molecules at bulk temperatures (~300-600 K) at which such species are thermally inaccessible [1]. The integration of atmospheric pressure NTPs with heterogeneous catalysts has recently gained considerable attention because of its potential to carry out transformations that are difficult or impossible using conventional thermal catalysis (e.g. N2, CH4, and CO2 activation) [2], and in modular units powered by renewable electricity. The synergy between plasma and catalyst originates from their interactions at the interface. Therefore, to better control the plasma-catalyst coupling, it is essential to understand the fundamental behavior of the underlying plasma-catalytic surface interactions.
Background: Nitrogen (N2) activation has attracted substantial interest in the plasma catalysis community because of the ability of plasma to activate the strong dinitrogen triple bond [3]. However, the nature of the nitrogen species involved in plasma-catalytic N2 chemistry is currently not well-understood. It has been postulated that plasma activation of N2 via vibrational or electronic excitation enhances the dissociative sticking probability of N2 on metal surfaces, thereby enhancing rates of generation of surface-bound N [4]. Other evidence points to atomic N created in a plasma-phase as a significant contributor to adsorbed N [5]. However, my preliminary results suggest that the types of adsorbed nitrogen species relevant to plasma catalysis may be richer than previously thought. Using in-situ polarization-modulation infrared reflection absorption spectroscopy (PM-IRAS) to probe surface-adsorbed nitrogen species during plasma jet impingement of plasma-activated N2 species to ambient-temperature metal (Ni, Pd, Cu, Ag, and Au) surfaces, I observed a vibrational band centered at ~2200 cm-1. The position of this band could be consistent with surface-bound N2. However, the intensity of the feature, and the temperature at which it is observed, are inconsistent with this assignment.
Proposed Research: An intriguing alternative is that the feature reflects a polynuclear nitrogen species accessed through the combined interaction of NTP and surface. In particular, azide, or N3, exists as a free radical and as an anion in compounds with a variety of active metals. Thus, we hypothesize that this vibrational band observed at ~2200 cm-1 is associated with surface-adsorbed N3. However, many fundamental questions remain unanswered: (i) What are the identity (N2, N3, or other) and nature of this species? (ii) Is this species formed in the plasma first and subsequently trapped by the metal surface, or does the surface facilitate its formation? (iii) How reactive are these surface-adsorbed species toward other reactants? Existing in-situ spectroscopy techniques and new in-situ Raman spectroscopy will be used to unambiguously identify the surface nitrogen species. Also, quasi-in-situ x-ray photoelectron spectroscopy (XPS) techniques will be developed to explore the N3 formation mechanism. As a model reaction to probe the reactivity of the purported surface-adsorbed N3, we choose the azide-alkyne “click” chemistry, an industrially important reaction for the synthesis of pharmaceuticals, pesticides, and functional coatings [6].
Outcomes: Understanding the formation and stabilization of metastable N3 species on surfaces and their surface reactivity will lead to new strategies for controlling product selectivity in plasma-enhanced catalysis, by selectively trapping metastable intermediates through the proper selection and tuning of the plasma-catalyst system and operating conditions.
Research ObjectivesObjective 1: Clarify the identity of the surface-adsorbed nitrogen species with a characteristic vibrational feature at ~2200 cm-1. We hypothesize that this species is associated with surface-bound N3. We aim to investigate the identity of the nitrogen species using multiple experimental approaches. Also, we will construct a new in-situ Raman spectroscopy tool to unambiguously observe an N3 symmetric stretching mode that is Raman active but infrared-inactive. Finally, temperature-programmed desorption (TPD) will be performed to detect N3 (m/z = 42 amu) desorption from the metal surfaces using mass spectrometry (MS), and the simultaneous disappearance of the Raman band from N3 symmetric stretching mode.
Objective 2: Identify the key species involved in the formation of the species with the vibrational feature at ~2200 cm-1. We hypothesize that the N3 formation is a two-step process: (1) deposition of atomic N on the metal surface and (2) addition of plasma-activated N2 to surface atomic N to form N3. We aim to understand what plasma-phase species are involved in the formation of the surface-adsorbed nitrogen species at ~2200 cm-1 by correlating the formation of this species to specific plasma-phase species. This will be achieved using multi-modal in-situ PM-IRAS + optical emission spectroscopy (OES) + mass spectrometry (MS) to simultaneously detect surface-adsorbed species, plasma-activated species, and gas-phase species, respectively. To determine whether the deposition of atomic N on the metal surface is the first step in the N3 formation mechanism, as hypothesized, we will monitor the initial stages of N2 plasma exposure of the single crystals using quasi-in-situ XPS.
Objective 3: Determine the potential to exploit metastable surface-adsorbed N3 species for subsequent bond-forming reactions. We hypothesize that metastable surface-adsorbed N3 species will react with terminal alkynes to produce the corresponding 1,2,3-triazole. To test this hypothesis, we will monitor the conversion of this surface-adsorbed nitrogen species at ~2200 cm-1 during exposure to alkynes (acetylene, propyne) using both PM-IRAS and Raman spectroscopy. Potential gas-phase products will also be monitored using mass spectrometry. The AAC reaction will be investigated as a function of surface temperature (23 to 200 °C) on the different single-crystal surfaces.
[1] A. Bogaerts, X. Tu, J.C. Whitehead, G. Centi, L. Lefferts, O. Guaitella, F. Azzolina-Jury, H.-H. Kim, A.B. Murphy, W.F. Schneider, T. Nozaki, J.C. Hicks, A. Rousseau, F. Thevenet, A. Khacef, M. Carreon, The 2020 plasma catalysis roadmap, J. Phys. Appl. Phys. 53 (2020) 443001. https://doi.org/10.1088/1361-6463/ab9048.
[2] P. Mehta, P. Barboun, D.B. Go, J.C. Hicks, W.F. Schneider, Catalysis Enabled by Plasma Activation of Strong Chemical Bonds: A Review, ACS Energy Lett. 4 (2019) 1115–1133.
https://doi.org/10.1021/acsenergylett.9b00263.
[3] L.R. Winter, J.G. Chen, N2 Fixation by Plasma-Activated Processes, Joule. 5 (2021) 300–315.
https://doi.org/10.1016/j.joule.2020.11.009.
[4] K.H.R. Rouwenhorst, L. Lefferts, On the mechanism for the plasma-activated N2 dissociation on Ru surfaces, J. Phys. Appl. Phys. 54 (2021) 393002. https://doi.org/10.1088/1361-6463/ac1226.
[5] Y. Engelmann, K. van ’t Veer, Y. Gorbanev, E.C. Neyts, W.F. Schneider, A. Bogaerts, Plasma Catalysis for Ammonia Synthesis: A Microkinetic Modeling Study on the Contributions of Eley–Rideal Reactions, ACS Sustain. Chem. Eng. 9 (2021) 13151–13163. https://doi.org/10.1021/acssuschemeng.1c02713.
[6] S. Kantheti, R. Narayan, K.V.S.N. Raju, The impact of 1,2,3-triazoles in the design of functional coatings, RSC Adv. 5 (2015) 3687–3708. https://doi.org/10.1039/C4RA12739K.
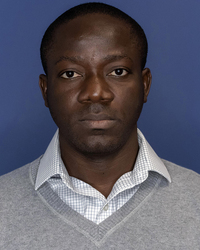
Agboola Suleiman
Chemical and Biomolecular Engineering
Faculty Advisor: Ruilan Guo
Discovery of High-Performance Gas Separation Polymer Membranes Assisted by Machine Learning
Membrane-based separation technologies have great promise to dramatically drive down the energy, carbon footprint, and water intensity of traditional thermally driven separation processes such as cryogenic distillation.[1, 2] Currently, the development of novel polymeric membrane materials for gas separation largely involves tuning chemical structures or incorporating groups that either have a higher affinity for specific gases or increase free volume for higher diffusivity. This process is largely informed by theoretical understandings, experience, and intuition but could lead to unintentional screening out of high-performance candidates. Also, this Edisonian process is frequently based on trial and error for achieving desirable microstructures inevitably leading to very long material development cycles. Therefore, machine learning (ML)-centered approaches that link the gas permeabilities and selectivities of polymers to their chemical descriptors have been recently leveraged to aid this hit-or-miss process and hasten the development of next-generation polymers for gas separation.[3, 4] [5, 6]
In this collaborative study between MONSTER and Guo labs, we seek to develop novel polymeric materials that break through the existing Robeson upper-bound trade-off lines for gas separation. To do this, ML tools would be deployed to identify promising polymers, some of these polymers would then be synthesized, characterized, and used to experimentally validate their gas separation predictions. Emphasis would also be placed on coming up with non-fluorinated materials that comply with recently tightened state and federal environmental laws to aid the industrial application of these materials. This study is anticipated to provide further insights and strategic direction toward the discovery of high-performance target polymers for gas separation.
Research ObjectivesThe overarching objective of this project is to design high-performance target polymers for separation of gases by
- developing machine learning models from reported gas separation polymers and using the models to predict the separation performance of polymers that were reported for applications other than gas separation
- selecting some of the best-performing polymers from ML models would be chosen based on the predicted gas separation performance, availability, and cost of their commercial monomers, and envisioned ease of synthesis of the polymers
- synthesizing the polymers and fabricating them into membranes
- conducting gas permeation tests, material characterization and validating the models with a view to optimizing them or adjusting polymer synthesis routes.
[1] Sanders, D.E., et al., Energy-efficient polymeric gas separation membranes for a sustainable future: A review. Polymer, 2013. 54(18): p. 4729-4761.
[2] Park, H.B., et al., Maximizing the right stuff: The trade-off between membrane permeability and selectivity. Science, 2017. 356(6343): p. eaab0530.
[3] Audus, D.J. and J.J. de Pablo, Polymer Informatics: Opportunities and Challenges. ACS Macro Lett, 2017. 6(10): p. 1078-1082.
[4] Chen, G., et al., Machine-Learning-Assisted De Novo Design of Organic Molecules and Polymers: Opportunities and Challenges. Polymers (Basel), 2020. 12(1): p. 163.
[5] Barnett, J.W., et al., Designing exceptional gas-separation polymer membranes using machine learning. Science Advances, 2020. 6(20): p. eaaz4301.
[6] Yang, J., et al., Machine learning enables interpretable discovery of innovative polymers for gas separation membranes. Sci Adv, 2022. 8(29): p. eabn9545.